About Our Research:
We are interested in sub-nanometer scale (< 10 nm) metallic particles, because they show different physical and chemical properties from their bulk counterparts. A fundamental issue in nanoparticle science in this size regime is to understand how their fundamental properties (e.g. charge separation, transport, and recombination; electronic transition and relaxation; and chemical & biological reactivities) change with their sizes, core compositions, and surface-protecting
ligands. Grounded in this issue, currently our research interests are in three areas:
-
-
-
I. Synthesis, Characterization, and Functionalization of Metallic and Alloyed Nanoparticles for Catalysts and Sensing Materials.
Surface process is an important step in catalysis and the development of sensors. For example, the overall rate of a catalytic reaction is proportional to the active surface area of a catalyst; the current density of electrochemical electron transfer depends on the total electrode area; and the area of a sensing site also relates to the sensitivity and detection limit of a sensor. Therefore, development and characterization of materials with a high surface-to-volume
ratio are desired for efficient catalytic reactions and sensing capabilities. Because nanoparticles have much higher surface-to-volume ratios than their bulk counterparts, they are promising as materials for catalysts and sensors.
It has been known that the electronic structure (i.e. density of state) of metal clusters smaller than ~10 nm (diameter) is different from that of corresponding bulk metals. Because a small number of atoms are involved in the formation of electronic bands in a nanoparticle, electrons are greatly localized in the valence band, and the narrow width of valence band is formed. In addition, the pressure towards the core center generated by the surface curvature of a
small nanoparticle induces a significant reduction of the lattice constant. The low lattice constant results in a shift of the d-band to higher energy states, which enhances the surface reactivity. Therefore, nanoparticles can have unique reactivity, which is more efficient (or even not shown in) than their bulk materials.
For synthesis, we adopt the literature methods (e.g. Brust's synthesis for gold nanoparticles), and modify them for our own purposes. As shown below, the synthesized nanoparticles are characterized by a variety of analytical techniques. For example, electrochemistry gives information on the charging property of nanoparticles, dynamics of electron transfer, etc. Functionalization of nanoparticles is directed toward applications in catalysts and sensors.
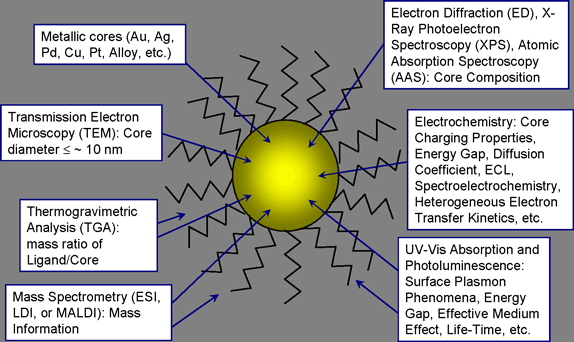
Figure 1. Characterization of nanoparticles
II. Exploration of Alloyed Nanoparticles as Fuel Cell Catalysts.
Fossil fuels (oil, coal, and natural gas) are a non-renewable energy source, and provide over 85% of the energy that we need. However, the amount of fossil fuels in the earth is limited, and our consumption of fossil fuels is increasing in every year. Nowadays demands for the development of alternative and renewable energy sources, such as fuel cells, is higher and higher.
Electrochemical reactions occurring from most typical hydrogen-oxygen fuel cells are as follows:

At both electrodes, the involved sequential processes are dissolution of H2 or O2 at the gas-electrolyte interface, diffusion of dissolved H2 and O2 to the catalyst surface, electrochemical catalytic redox reactions of dissolved H2 and O2, and proton transfer through the electrolyte (Figure 2). The above issues play important roles in overall fuel cell efficiency, and must be optimized.
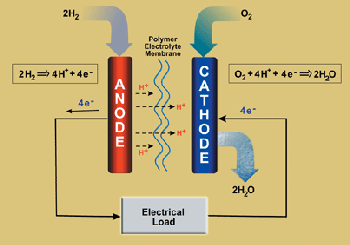
Figure 2. Schematic diagram of a typical fuel cell processes (courtesy of U.S. environmental protection agency, http://www.epa.gov/fuelcell/gifs/fuel-cell.gif)
In this project, we study the performance of electrocatalysts for fuel cells. We are interested in the development of replacements of Pt-related nanoparticle catalysts. Despite of its excellent catalytic performance, Pt is too expensive to commercialize Pt-based fuel cells. In addition, the power density of the current Pt-based fuel cell is still too low to meet our demands. Therefore, it is necessary to discover alternative catalysts for future fuel cells. A wide
variety of alloyed metal nanoparticles are considered as catalysts for key catalytic reactions in fuel cells: hydrogen oxidation; oxygen reduction; and electro-oxidation of methanol, formaldehyde, and formic acid. Because the overall efficiency of a fuel cell is not governed by only the performance of electrocatalysts, it is crucial to characterize fuel cell electrodes, which contains an assembly of nanoparticle catalysts supported on activated carbons (Vulcan 72) with a binder (polytetrafluoroethylene,
PTFE), in contact with the electrolyte. Measuring the current density (or open-circuit potential) from this electrode system will show how the active layers of carbon-supported nanoparticle catalysts work efficiently under fuel cell conditions.
III. Sensor Applications of Functionalized Metal Nanoparticles.
The development of sensors that can detect specific biomolecules and hazardous chemicals is important in clinical diagnostics, forensic chemistry, environmental investigations, and biological warfare agent detection. The use of metal nanoparticles as sensor materials is highly promising, because the local microenvironment of metal nanoparticles can be controlled by the cross-linking elements, and may lead to specific and selective interactions with substrates.
Specific interaction between the analytes and the organic shell functional groups attached on the nanoparticle surface can also enhance sensitivity and increase selectivity.
In this project, we develop three different types of sensors. First, we are interested in gas (or vapor) sensors (chemiresistors) using Au nanoparticle films, where electrons are transported by electron hopping. If the metal nanoparticle film swells by a specific gas (or vapor), the core-to-core distance between nanoparticles increases and the film conductivity (sEL) decreases, depending on the extent of swelling (Figure 3). To detect a target gas, metal
nanoparticles are functionalized with molecules that can swell mainly with a target gas. For example, poly(ethylene glycol) can swell by CO2. Then the gas sensor is constructed by preparing a functionalized nanoparticle film on the interdigitated array (IDA) electrode. Optimization of selectivity and sensitivity will be made by differentiating film structures, core sizes, and dielectric permittivity of an interparticle medium. Initial consideration are given to detection of toxic gases (or vapors),
such as CO, HCN, and gases from chemical weapons (tabun, sarin, soman, etc.).
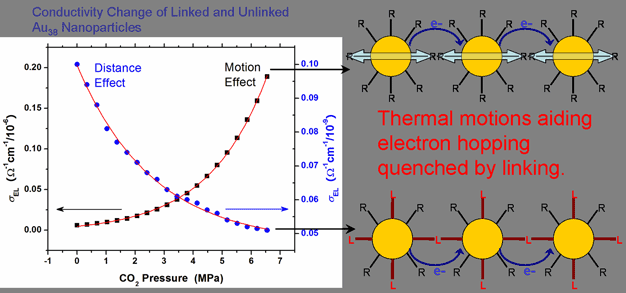
Figure 3. Conductivity change of Au38 nanoparticle films as a function of CO2 pressure
Second, we aim at exploitation of low cost biosensors with high sensitivity and selectivity based on conductivity. It is possible that Au nanoparticle assemblies are formed in the gap between the electrodes by antigen-antibody interactions. Probe antibodies are immobilized on Au nanoparticles as well as in the electrode gap. Then the specific antigen-antibody reaction proceed in the presence of antibody-immobilized Au nanoparticles and antigens, so that the
assembly of antigen-antibody cross-linked Au nanoparticles is formed (Figure 4. B), and sEL can be measured. However, no assembly of Au nanoparticles is formed in the absence of specific antigens (Figure 4. A), and no conduction would occur.
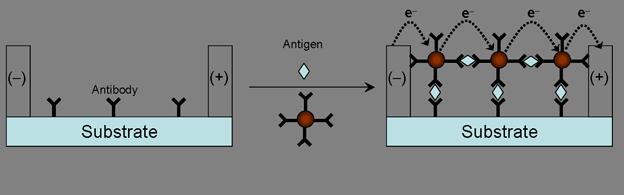
Figure 4. Schematic diagram of electrical detection of antigens with Au nanoparticle probes.
Third, the above sensing scheme can be expanded to the detection of heavy metal ions, such as Pb2+, Cd2+, and Hg2+, in water. The surface of nanoparticle is functionalized by binding ligands, such as crown ether derivatives, and the monolayer of functionalized nanoparticles is formed at the substrate. Depending on the amount of bound heavy metal ions, the fundamental properties (e.g. conductivity, absorbance, luminescence, molecular vibration,
etc) of nanoparticle monolayer may change. This change allows to do the convenient quantitative analysis of heavy metal ions in water with low cost.

Figure 5. Schematic view of a sensor for heavy metal detection.
|